Introduction to Microscope Objectives Microscope objectives are perhaps the most important components of an optical microscope because they are responsible for primary image formation and play a central role in determining the quality of images that the microscope is capable of producing. Objectives are also instrumental in determining the magnification of a particular specimen and the resolution under which fine specimen detail can be observed in the microscope.
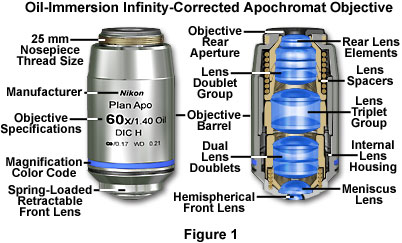 The objective is the most difficult component of an optical microscope to design and assemble, and is the first element that light encounters as it proceeds from the specimen to the image plane. Objectives derive their name from the fact that they are, by proximity, the closest component to the object (specimen) being imaged. Major microscope manufacturers offer a wide range of objective designs, which feature excellent optical characteristics under a wide spectrum of illumination conditions and provide various degrees of correction for the primary optical aberrations. The objective illustrated in Figure 1 is a 60x oil immersion apochromat, which contains 15 optical elements that are cemented together into three groups of lens doublets, a lens triplet group, and three individual internal single-element lenses. The objective also has a hemispherical front lens and a meniscus second lens, which work synchronously to assist in capturing light rays at high numerical aperture with a minimum of spherical aberration. As is the case with most oil immersion objectives, the apochromat illustrated in Figure 1 is equipped with a spring-loaded retractable nosecone assembly that protects the front lens elements and the specimen from collision damage. Internal lens elements are carefully oriented and tightly packed into a tubular brass housing that is encapsulated by the objective barrel. Specific objective parameters such as numerical aperture, magnification, optical tube length, degree of aberration correction, and other important characteristics are imprinted or engraved on the external portion of the barrel. Although the objective featured in Figure 1 is designed to operate utilizing oil as the imaging medium between the objective front lens and specimen, other objectives have front lens elements that allow them to be use either in air or immersed in water, glycerin, or a other specialized hydrocarbon-based oils. Modern objectives, made up of many glass elements, have reached a high state of quality and performance, with the extent of correction for aberrations and flatness of field determining the usefulness and cost of an objective. Construction techniques and materials used to manufacture objectives have greatly improved over the course of the past 100 years. Today, objectives are designed with the assistance of Computer-Aided-Design (CAD) systems using advanced rare-element glass formulations of uniform composition and quality having highly specific refractive indices. The enhanced performance that is demonstrated using these advanced techniques has allowed manufacturers to produce objectives that are very low in dispersion and corrected for most of the common optical artifacts such as coma, astigmatism, geometrical distortion, field curvature, spherical and chromatic aberration. Not only are microscope objectives now corrected for more aberrations over wider fields, but image flare has been dramatically reduced with a substantial increase in light transmission, yielding images that are remarkably bright, sharp, and crisp. The least expensive (and most common) objectives, employed on a majority of laboratory microscopes, are the achromatic objectives. These objectives are corrected for axial chromatic aberration in two wavelengths (blue and red; about 486 and 656 nanometers, respectively), which are brought into a single common focal point. Furthermore, achromatic objectives are corrected for spherical aberration in the color green (546 nanometers; see Table 1). The limited correction of achromatic objectives can lead to substantial artifacts when specimens are examined and imaged with color microscopy and photomicrography. If focus is chosen in the green region of the spectrum, images will have a reddish-magenta halo (often termed residual color). Achromatic objectives yield their best results with light passed through a green filter (often an interference filter) and using black and white film when these objectives are employed for photomicrography. The lack of correction for flatness of field further hampers achromat objectives. In the past few years, most manufacturers have begun providing flat-field corrections for achromat objectives and have given these corrected objectives the name of plan achromats.
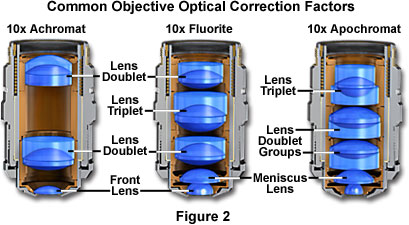 The next higher level of correction and cost is found in objectives called fluorites or semi-apochromats (illustrated by center objective in Figure 2), named for the mineral fluorite, which was originally used in their construction. Figure 2 depicts the three major classes of objectives: The achromats with the least amount of correction, as discussed above; the fluorites (or semi-apochromats) that have additional spherical corrections; and, the apochromats that are the most highly corrected objectives available. The objective positioned on the far left in Figure 2 is a 10x achromat, which contains two internal lens doublets and a front lens element. Illustrated in the center of Figure 2 is a 10x fluorite objective having several lens groups including two doublets and a triplet, in addition to a hemispherical front lens and a secondary meniscus lens. On the right in Figure 2 is a 10x apochromat objective that also contains multiple lens groups and single elements. Although similar in construction to fluorite objectives, the lenses have different thicknesses and curvatures and are arranged in a configuration that is unique to apochromat objectives. Objective Correction for Optical Aberration
Objective Type |
Spherical Aberration |
Chromatic Aberration |
Field Curvature |
Achromat |
1 Color |
2 Colors |
No |
Plan Achromat |
1 Color |
2 Colors |
Yes |
Fluorite |
2-3 Colors |
2-3 Colors |
No |
Plan Fluorite |
3-4 Colors |
2-4 Colors |
Yes |
Plan Apochromat |
3-4 Colors |
4-5 Colors |
Yes | |
|

|

|

|
Table 1 Fluorite objectives are produced from advanced glass formulations that contain materials such as fluorspar or newer synthetic substitutes. These new formulations allow for greatly improved correction of optical aberration. Similar to the achromats, the fluorite objectives are also corrected chromatically for red and blue light. In addition, the fluorites are also corrected spherically for two or three colors instead of a single color, as are achromats. The superior correction of fluorite objectives compared to achromats enables these objectives to be made with a higher numerical aperture, resulting in brighter images. Fluorite objectives also have better resolving power than achromats and provide a higher degree of contrast, making them better suited than achromats for color photomicrography in white light. Interactive Java Tutorial Numerical Aperture
Investigate how the size of the light cone entering the objective front lens changes with the objective numerical aperture value. The highest level of correction (and expense) is found in apochromatic objectives, illustrated in Figures 2 and 3. Apochromats represent the most highly corrected microscope lenses currently available, and their high price reflects the sophisticated design and careful assembly required in their manufacture. In Figure 3, we compare lens elements in a series of apochromatic objectives ranging from 10x to 100x in magnification. The lower power apochromat objectives (10x and 20x) have a longer working distance and the overall objective length is shorter than in higher power (40x and 100x) apochromat objectives. Apochromats are corrected chromatically for three colors (red, green, and blue), almost eliminating chromatic aberration, and are corrected spherically for either two or three wavelengths (see Table 1). Apochromatic objectives are the best choice for color photomicrography in white light. Because of their high level of correction, apochromat objectives usually have, for a given magnification, higher numerical apertures than do achromats or fluorites. Many of the newer high-performance fluorite and apochromat objectives are corrected for four (dark blue, blue, green, and red) or more colors chromatically and four colors spherically.
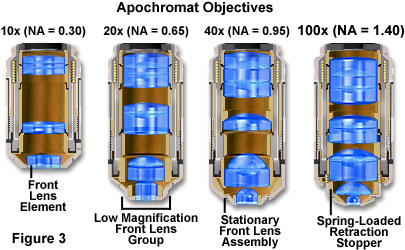 All three types of objectives suffer from pronounced field curvature and project images that are curved rather than flat, an artifact that increases in severity with higher magnification. To overcome this inherent condition arising from curved lens surfaces, optical designers have produced flat-field corrected objectives, which yield images that are in common focus throughout the viewfield. Objectives that have flat-field correction and low distortion are called plan achromats, plan fluorites, or plan apochromats, depending upon their degree of residual aberration. Such correction, although expensive, is quite valuable in digital imaging and conventional photomicrography. Interactive Java Tutorial Field Curvature
A simple lens focuses image points from an extended flat object, such as a specimen on a microscope slide, onto a spherical surface resembling a curved bowl. Explore how field curvature aberrations degrade images observed in the microscope. Uncorrected field curvature is the most severe optical aberration that occurs in fluorite (semi-apochromat) and apochromat objectives, and it was tolerated as an unavoidable artifact for many years. During routine use, the viewfield would have to be continuously refocused between the center and the edges to capture all specimen details. The introduction of flat-field (plan) correction to objectives perfected their use for photomicrography and video microscopy, and today these corrections are standard in both general use and high-performance objectives. Correction for field curvature adds a considerable number of lens elements to the objective as illustrated in Figure 4 with a simple achromat. The uncorrected achromat on the left in Figure 4 contains two lens doublets, in addition to a simple thin-lens front element. In contrast, the corrected plan achromat on the right in Figure 4 contains three lens doublets, a central lens triplet group, and a meniscus lens positioned behind the hemispherical front lens. Plan correction, in this instance, has led to the addition of six lens elements bundled into more sophisticated lens groupings, which dramatically increases the optical complexity of the objective. The significant increase in lens elements for plan correction also occurs with fluorite and apochromat objectives, frequently resulting in an extremely tight fit of lens elements (see Figure 1) within the internal objective sleeve. In general, plan objectives corrected for field curvature sacrifice a considerable amount of free working distance, and many of the high-magnification versions have a concave front lens, which can be extremely difficult to clean and maintain.
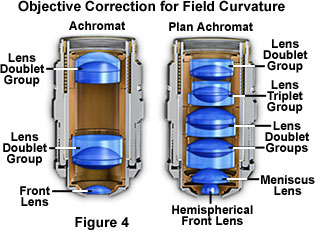 Older objectives generally have lower numerical apertures, and are subject to an aberration termed chromatic difference of magnification that requires correction by the use of specially designed compensating oculars or eyepieces. This type of correction was prevalent during the reign of fixed tube length microscopes, but is not necessary with modern infinity-corrected objectives and microscopes. In recent years, modern microscope objectives have their correction for chromatic difference of magnification either built into the objectives themselves (Olympus and Nikon) or corrected in the tube lens (Leica and Zeiss). The intermediate image in an infinity-corrected system appears at the reference focal length (formerly, the optical tube length) behind the tube lens in the optical pathway. This length varies between 160 and 250 millimeters, depending upon design constraints imposed by the manufacturer. The magnification of an infinity-corrected objective is calculated by dividing the reference focal length by the focal length of the objective lens. In most biological and petrographic applications, a cover glass is utilized in mounting the specimen, both to protect the integrity of the specimen and to provide a clear window for observation. The cover glass acts to converge the light cones originating from each point in the specimen, but also introduces chromatic and spherical aberration (and consequent loss of contrast) that must be corrected by the objective. The degree to which light rays are converged is determined by the refractive index, dispersion, and thickness of the cover glass. Although the refractive index should be relatively constant within a batch of cover glasses, the thickness can vary between 0.13 and 0.22 millimeters. Another concern is the aqueous solvent or excess mounting medium that lies between the specimen and cover glass in wet or thickly mounted preparations. For example, in physiological saline whose refractive index is significantly different from that of the coverslip, the objective must focus through a layer of water only a few microns thick, leading to significant aberrations and a deviation of the point spread function that is no longer symmetrical above and below the focal plane. These factors add to the effective variations in refractive index and thickness of the coverslip and are very difficult for the microscopist to control. The imaging medium between the objective front lens and the specimen coverslip is also very important with respect to correction for spherical aberration and coma in the design of lens elements for objectives. Lower power objectives have relatively low numerical apertures and are designed to be used dry with only air as the imaging medium between the objective front lens and the cover glass. The maximum theoretical numerical aperture obtainable with air is 1.0, however in practice it is virtually impossible to produce a dry objective with a numerical aperture above 0.95. The effect of cover glass thickness variation is negligible for dry objectives having numerical apertures less than 0.4, but such deviation becomes significant at numerical apertures exceeding 0.65, where fluctuations as small as 0.01 millimeter can introduce spherical aberration. This poses problems with high-power apochromats, which must use very short working distances in air and contain sensitive corrections for spherical aberration that tend to make it difficult to obtain sharp images. Interactive Java Tutorial Cover Glass Correction
Investigate how internal lens elements in a high numerical aperture dry objective may be adjusted to correct for fluctuations in cover glass thickness. To remedy this, many high-performance apochromat dry objectives are fitted with correction collars, which allow adjustment to correct for spherical aberration by correcting for variations in cover glass thickness (see Figure 5). Optical correction for spherical aberration is produced by rotating the collar, which causes two of the lens element groups in the objective to move either closer together or farther apart. The objective on the left in Figure 5 has had the correction collar adjusted for a cover glass thickness of 0.20 mm by bringing the adjustable lens elements very close together. In contrast, the objective on the right in Figure 5 has the adjustable lens elements separated by a rather large distance to compensate for very thin cover glasses (0.13 mm). A majority of the correction collar objectives designed for upright transmitted light microscopy have an adjustment range for cover glass thickness variations between 0.10 and 0.23 millimeters. Many of the specialized phase contrast objectives designed for observing tissue culture specimens with an inverted microscope have an even broader compensation range of 0 to 2 millimeters. This allows specimens to be viewed through the bottom of most culture vessels, which often have dramatic thickness fluctuations in this size range. Uncovered specimens, such as blood smears, can also be observed with correction collar objectives when the adjustment is set to 0 to account for the lack of a cover glass. High numerical aperture dry objectives lacking a correction collar often produce images that are inferior to those of lower numerical aperture objectives where cover glass thickness is of less concern. For this reason, it is often prudent to choose a lower magnification (and numerical aperture) objective in order to obtain superior contrast without the accompanying artifacts introduced by cover glass fluctuations. As an example, a 40x objective having a numerical aperture of 0.65 may be able to produce better images with sharper contrast and clarity than a 60x-0.85 numerical aperture objective, even though the resolving power of the higher magnification objective is theoretically greater.
The standard thickness for cover glasses is 0.17 millimeters, which is designated as a number 1?/B> cover glass. Unfortunately, not all 1?cover glasses are manufactured to this close tolerance (they range from 0.16 to 0.19 millimeters) and many specimens have media between them and the cover glass. Compensation for cover glass thickness can be accomplished by adjusting the mechanical tube length of the microscope, or (as previously discussed) by the utilization of specialized correction collars that change the spacing between critical elements inside the objective barrel. The correction collar is utilized to adjust for these subtle differences to ensure the optimum objective performance. Proper utilization of objective lenses with correction collars demands that the microscopist is experienced and alert enough to reset the collar using appropriate image criteria. In most cases, focus may shift and the image may wander during adjustment of the correction collar. Use the steps listed below to make small incremental adjustments to an objective¡¯s correction collar while observing changes in the specimen image.
- Position the correction collar so that the indicator mark on the objective barrel coincides with the 0.17 millimeter scale mark engraved on the collar housing.
- Place a specimen on the stage and focus the microscope on a small specimen feature.
- Rotate the correction collar very slightly and re-focus the objective to determine if the image has improved or degraded. Due to the fact that most specimen preparations suffer from cover glass/media sandwiches that are too thick, start the rotation experiment by trying larger compensation values (0.18-0.23) first.
- Repeat the previous step to determine if the image is improving or degrading as the correction collar is turned in a single direction.
- If the image has degraded, follow the same steps and rotate the correction collar in the opposite direction (toward lower values) to find the position offering optimum resolution and contrast.
Objective numerical aperture can be dramatically increased by designing the objective to be used with an immersion medium, such as oil, glycerin, or water. By using an immersion medium with a refractive index similar to that of the glass coverslip, image degradation due to thickness variations of the cover glass are practically eliminated whereby rays of wide obliquity no longer undergo refraction and are more readily grasped by the objective. Typical immersion oils have a refractive index of 1.51 and a dispersion similar to that of glass coverslips. Light rays passing through the specimen encounter a homogeneous medium between the coverslip and immersion oil and are not refracted as they enter the lens, but only as they leave its upper surface. It follows that if the specimen is placed at the aplanatic point of the first objective lens, imaging by this portion of the lens system is totally free of spherical aberration.
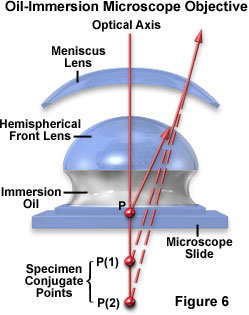 The general design of a practical oil immersion objective includes a hemispherical front lens element, followed by a positive meniscus lens and a doublet lens group. Presented in Figure 6 are the aplanatic refractions that occur at the first two lens elements in a typical apochromatic oil immersion objective. The specimen is sandwiched between the microscope slide and cover glass at point P, the aplanatic point of the hemispherical lens element. Light rays refracted at the rear of the hemispherical lens appear to proceed from point P(1), which is also the center of curvature for the first surface of the meniscus lens. The refracted light rays enter the meniscus lens along the radius of its first surface and experience no refraction at that surface. At the rear surface of the meniscus lens, light rays are refracted aplanatically, so they appear to diverge from point P(2). Refraction of the light rays at the surfaces of subsequent lens groups in the objective complete the convergence of light rays originating from point P, thus forming the intermediate image. Properly designed oil immersion objective lenses also correct for chromatic defects that are introduced by the first two lens elements, while introducing a minimum amount of spherical aberration. The fact that the light cone is partially converged before entering the first lens element aids in the control of spherical aberration. It should be noted that employing an oil immersion objective without the application oil between the coverslip and first lens element results in defective images. This due to refraction that occurs at the surface of the front lens, which introduces spherical aberration that cannot be corrected by subsequent lens components within the objective. Interactive Java Tutorial Immersion Oil and Refractive Index
Explore how variations in the refractive index of the imaging medium effect the ability of an objective to capture light rays emanating from the specimen. The advantages of oil immersion objectives are severely compromised if the wrong immersion fluid is utilized. Microscope manufacturers produce objectives with tight tolerances to refractive index and dispersion, which require matching values in the liquid placed between the cover glass and objective front lens. It is advisable to employ only the oil intended by the objective manufacturer, and to not mix immersion oils between manufacturers to avoid unpleasant artifacts such as crystallization or phase separation. Objectives that use water and/or glycerin as an imaging medium are also available for applications with living cells in culture or sections of tissue immersed in physiological saline solution. Plan apochromat water immersion lenses are equipped with correction collars and numerical apertures up to 1.2, slightly less than their oil immersion counterparts. These objectives allow microscopists to focus through up to 200 microns of aqueous media and still retain excellent optical correction. The downside is that high numerical aperture water immersion lenses often cost many thousands of dollars and the image can still degrade when the objective is focused deeply through refractile tissue or cell parts. For more details on water, glycerin, and oil immersion objectives, visit the Molecular Expressions Microscopy Primer. |